space detector LISA
One guy studied physics and tried to refute the conclusions of the Theory of Relativity. He even tried to publish his research in the prestigious journal Physical Review Letters. It was deservedly shaved off by the reviewers and turned around by the editor of the magazine – the article was erroneous. And it’s good, because it would be awkward, to put it mildly. This guy was Albert Einstein.
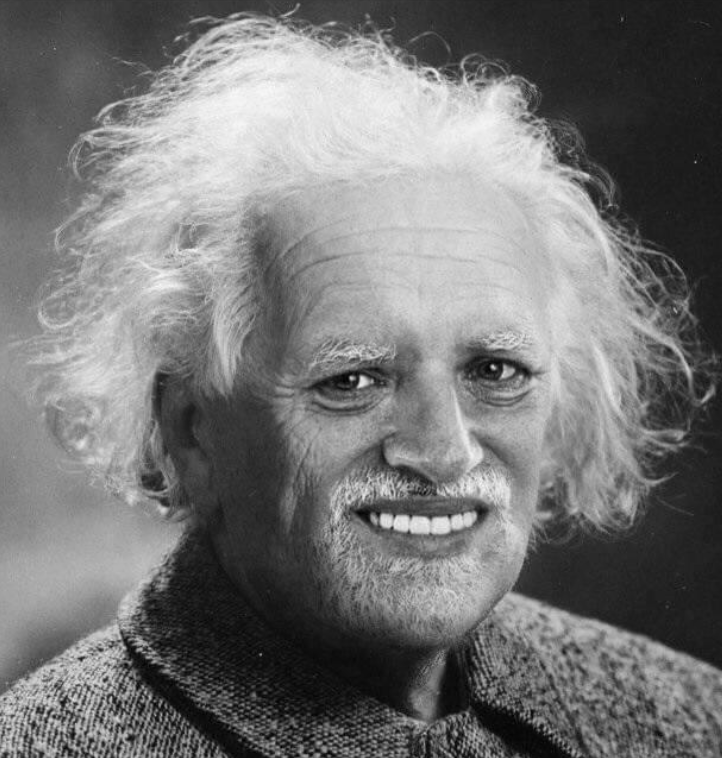
Indeed, the history of the discovery of gravitational waves is full of interesting twists and turns. Einstein himself discovered waves, and then abandoned his discovery (and then changed his point of view again). For 50 years after that, they were considered a mathematical artifact, until Feynman presented a very important argument in favor of their existence. For several years there was a battle of the best minds in the debate over the correctness of the argument, after which they began to come up with ways to observe them. The waves had already been “observed” experimentally in the 60s, and the article about the discovery caused a lot of noise. However, no one could confirm the discoveries, and they had to be “closed”, a tragedy for Weber, the author of the works. It took another 40 years and a lot of effort to build detectors that could actually observe them. A hundred years later, in 2015, we at LIGO recorded the first signal. Over the past five years, the number of events has exceeded 90, and we have seen mergers of black holes, and neutron stars, and pairs of a neutron star and a black hole.
Unfortunately, the LIGO-Virgo-Kagra detectors can only observe certain events – various noises greatly interfere with observations. In the coming years, construction will begin on a new underground detector, the Einstein Telescope, which will significantly increase the number of observed events (there will be so many that at any given time we will see several signals simultaneously). But he will not be able to observe low-frequency signals: this is due to the seismic activity of the Earth. There is only one way out: go into space. This is exactly what is planned in the LISA space detector. I’ll tell you about it today.
And if you want to learn more about the history of the discovery of gravitational waves, I highly recommend this article: https://arxiv.org/ftp/arxiv/papers/1810/1810.07994.pdf
A little about gravitational wave detectors
If you know how detectors work, feel free to skip this part.
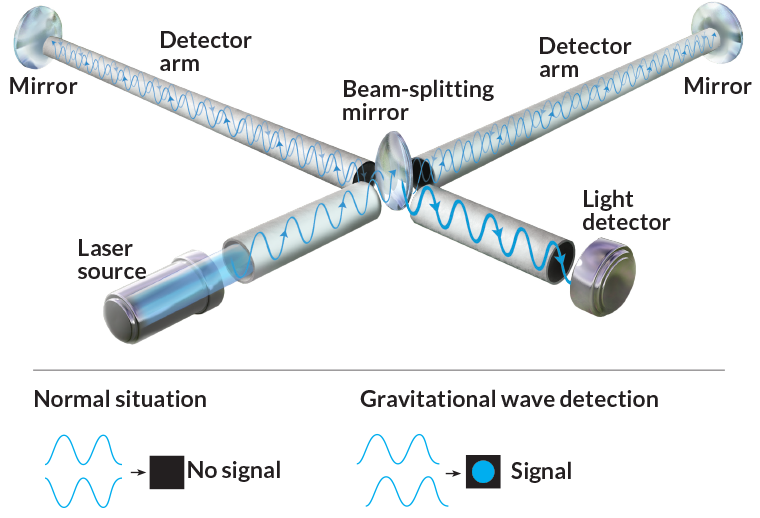
General relativity tells us that gravity is actually the curvature of spacetime. Bodies move in this curved space of time along the shortest distance. To us, this looks like a curvature of the trajectory, as in the picture below. The curvature of space-time leads to changes in the distances between objects.
Any mass and energy (!) bend space-time, so that not only massive bodies attract to themselves, but simply energy (for example, light) too.

Gravitational waves (GV) – small disturbances [метрики] space-time. They arise during the asymmetrical motion of massive bodies, for example, during the merger of two black holes. These disturbances propagate from the source at the speed of light, briefly altering the local curvature. As we found out above, curvature determines the distances between objects. That is, gravitational waves change the relative distance between two bodies: “compresses” or “expands” it.
The gravitational wave detector is designed to measure this change in distances using lasers. In its simplest form, the detector is a Michelson interferometer, where the detector arms are balanced so that, due to constructive interference, all light is reflected towards the source, and the second output of the beam splitter remains dark due to destructive interference.
When the GWs reach the detector, they stretch one arm and compress the other, which changes the interference pattern at the output of the interferometer and allows the signal to be recorded.
In the previous article, I explained that the GW detector is not a ruler, but a clock, i.e. measures the relative delay of light in the two arms caused by a gravitational wave. I also showed that the relative change in the phase of light when the metric changes by h_0 depends on the length of the detector L:
The light power at the photodiode is proportional to this phase, which explains why detectors are made so long (4 km LIGO, 10 km Einstein Telescope): this allows for increased sensitivity.
Difficulties in detectors
So, it would seem: the longer the shoulder length, the greater the sensitivity. Let's build longer detectors, what's the problem?
Two problems:
On Earth it is difficult to construct a straight line with a length of more than a couple of tens of kilometers: the Earth, unfortunately, is a ball. Therefore, in the future Cosmic Explorer detector in the USA with a length of 40 km, it will be necessary to straighten the curvature of the planet, cutting off the earth by several meters. Expensive and difficult. 40km is about the limit of what we can do.
The longer the detector, the lower the maximum signal frequency it can pick up. And on Earth, this puts an end to long detectors: at low frequencies below 1 Hz, the earth continuously shakes: earthquakes, volcanic eruptions, sea surf, human activity, etc. This interferes with even current detectors, and the future Einstein Telescope will be shoved 300m underground to hide it from shaking. But this is not enough: to isolate from the movement of the earth, long suspensions of 10-17 m are used. And all this will allow you to measure frequencies only above 5Hz.
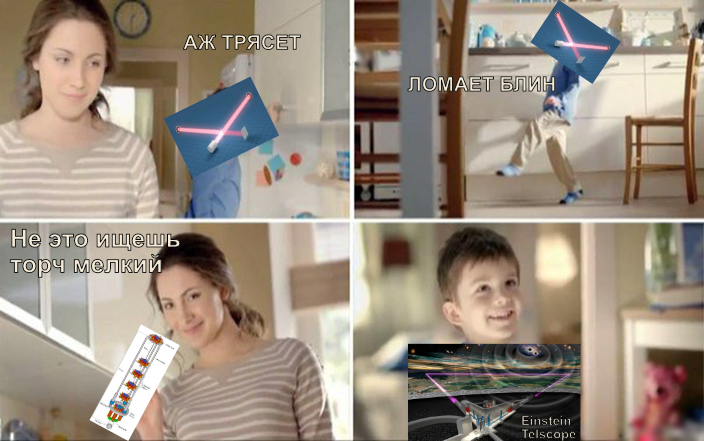
And at frequencies below 1Hz, I must say, there are a lot of interesting and important signals:
The same mergers of neutron stars or black holes can be seen months before the merger itself (since the frequency of GWs increases as they get closer, but initially they rotate at a very low frequency.
Mergers of supermassive black holes at the centers of galaxies
Mergers of supermassive black holes with masses tens of thousands of solar
Mergers of binary systems with large differences in mass (for example, 10,000 and 20 solar).
Gravity wave background
and who knows what else will come along!
By the way, you can read in detail about different sources in this guide to gravitational waves.
Solution: let's fly into space!
The solution to these problems is “simple”: let’s build the same detector, but in space. There you can make any arm length and measure very low frequency signals. At the same time, no people will crash into the detector, driving around the wasteland drunk (true story), and birds will not hammer into vacuum pipes, pretending to be a signal (also a true story).
So, I ask you to love and favor: LISA (Laser Interferometer Space Antenna).
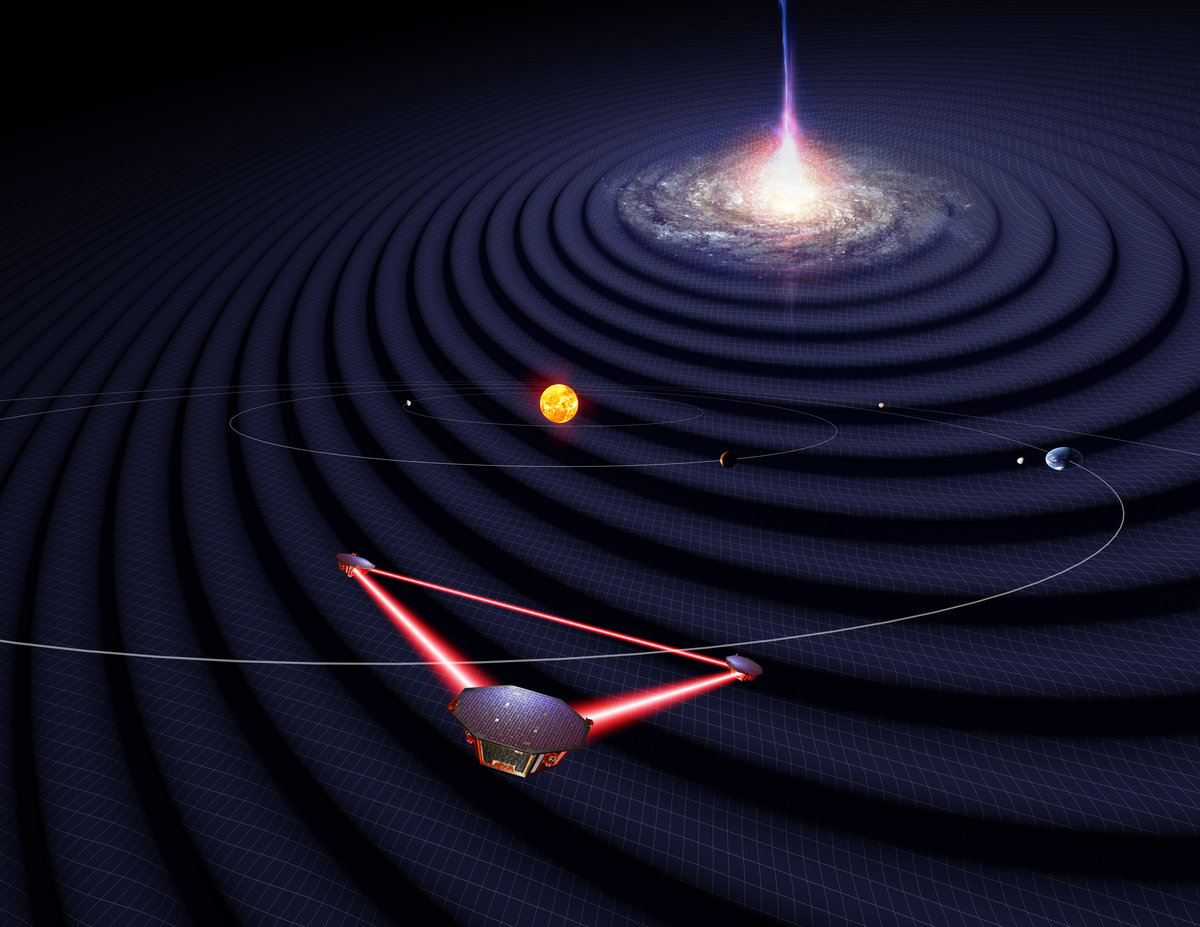
The detector will consist of three satellites that will fly in a triangular configuration in the Earth's orbit around the Sun. The laser beams will propagate from one satellite to the other, so that each pair of beams will form one interferometer (for a total of three).
The main feature: the length of the interferometer arm (i.e. the distance between the satellites) will be 2.5 million km. For comparison, the distance from the Earth to the Moon is about 380 thousand km.
This arm length will make it possible to register gravitational waves with frequencies between 10 μHz and 10 MHz.
But as you can imagine, there are a bunch of other problems with this approach. Let's figure it out.
Basic operating principle of LISA
Remember that gravitational waves change the distances between objects? So, LISA will be able to record changes in the length of the arm 50 times smaller than the size atom. I repeat: changes in length are 2.5 million km. by 1/50th the size of an atom. How is this even possible?!
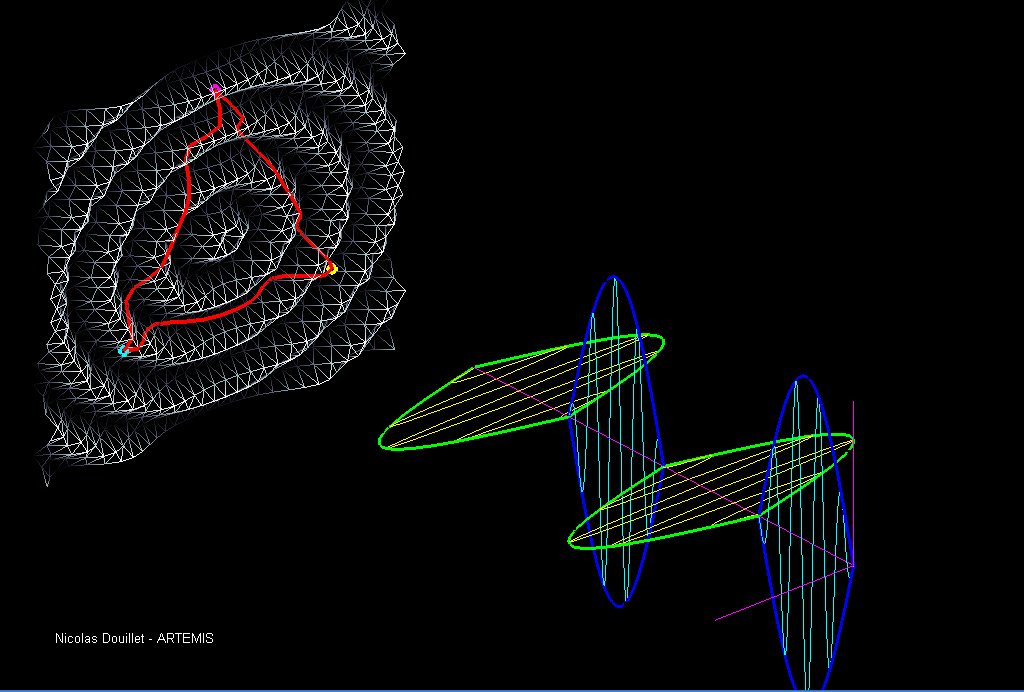
If you've read my past articles, you already know the answer: measured change length, not the length itself. A change in length leads to a time delay in the flight of the photon, and we can easily measure this delay with great accuracy: this is the essence of the interferometer. But how, you say, can satellites really be positioned with such precision? What about local noise, dust particles, fluctuations of the material itself, after all? This is where the main difficulty lies.
The most important thing: the distances between test masses that fly in free flight in orbit are measured. Test masses are small golden cubes. They are placed inside the satellite in a special container, but do not touch it and fly in inertial flight. The satellite adjusts its orbit to maintain its distance from the cubes. A laser beam is sent from one satellite to another, reflected from a cube there and returned back, interfering with another beam, measuring the delay.
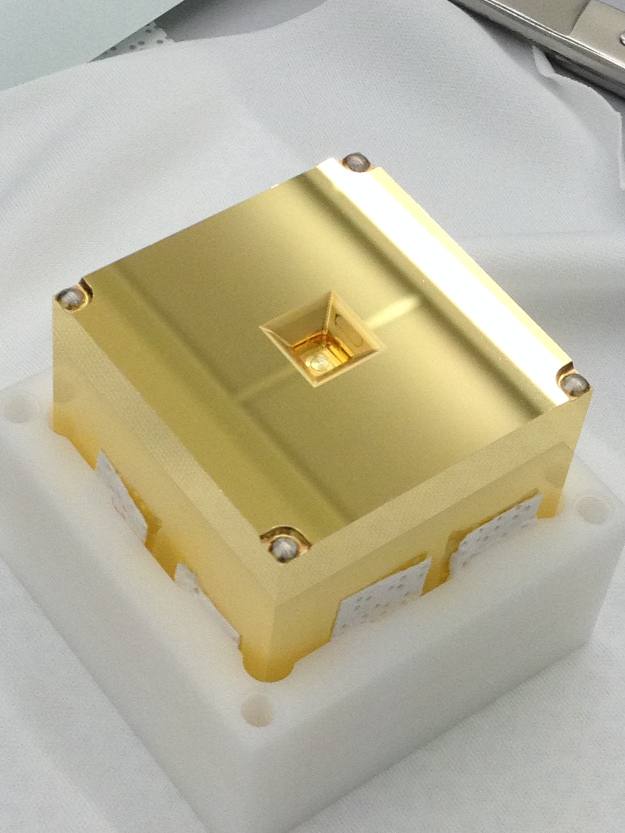
The orbit of satellites can be unstable: they can shift under the influence of external forces and disturbances. This happens very slowly, and they slightly change the orbits of the cubes through electrostatic action. The satellites continuously measure the distance between each other (to within cm using conventional ranking methods) and take this into account when creating an effect on the cube (to ensure that they are not gravitational waves). Thus, interferometers always see changes in length at very low frequencies, and gravitational waves appear as modulations of these signals.
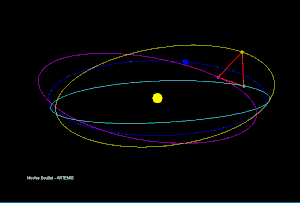
The devil is in the details
Does the above seem very complicated? Hold my beer. You will come away from this part of the article convinced that this is impossible.
A laser beam is not a beam in the literal sense of the word. Of course, when you pick up a laser pointer, it seems like the laser beam is essentially a straight line. But if you played with them, shining
neighbors through the windowsinto the trees somewhere in the distance, you saw that the small spot of the laser coming out of the pointer became quite large in the distance. This is a fundamental property of light: the farther (from the focus, where its diameter is minimal) the beam propagates, the wider it becomes. Let's say your laser pointer at a distance of 1 m will have a diameter of 1 mm, 100 m – 2 cm, 10 km – 2 m, and at a distance of 2.5 million kilometers – about 500km. Let me remind you that the size of the cube is several cm. It is easy to calculate that about 1/25000000000000 of the light emitted by your pointer will fall on the cube. If the pointer has a power of 1 mW, then only about a hundred photons per second will reach the cube. But they still need to be reflected and fly the same distance back: in the end, you will lose all the light.
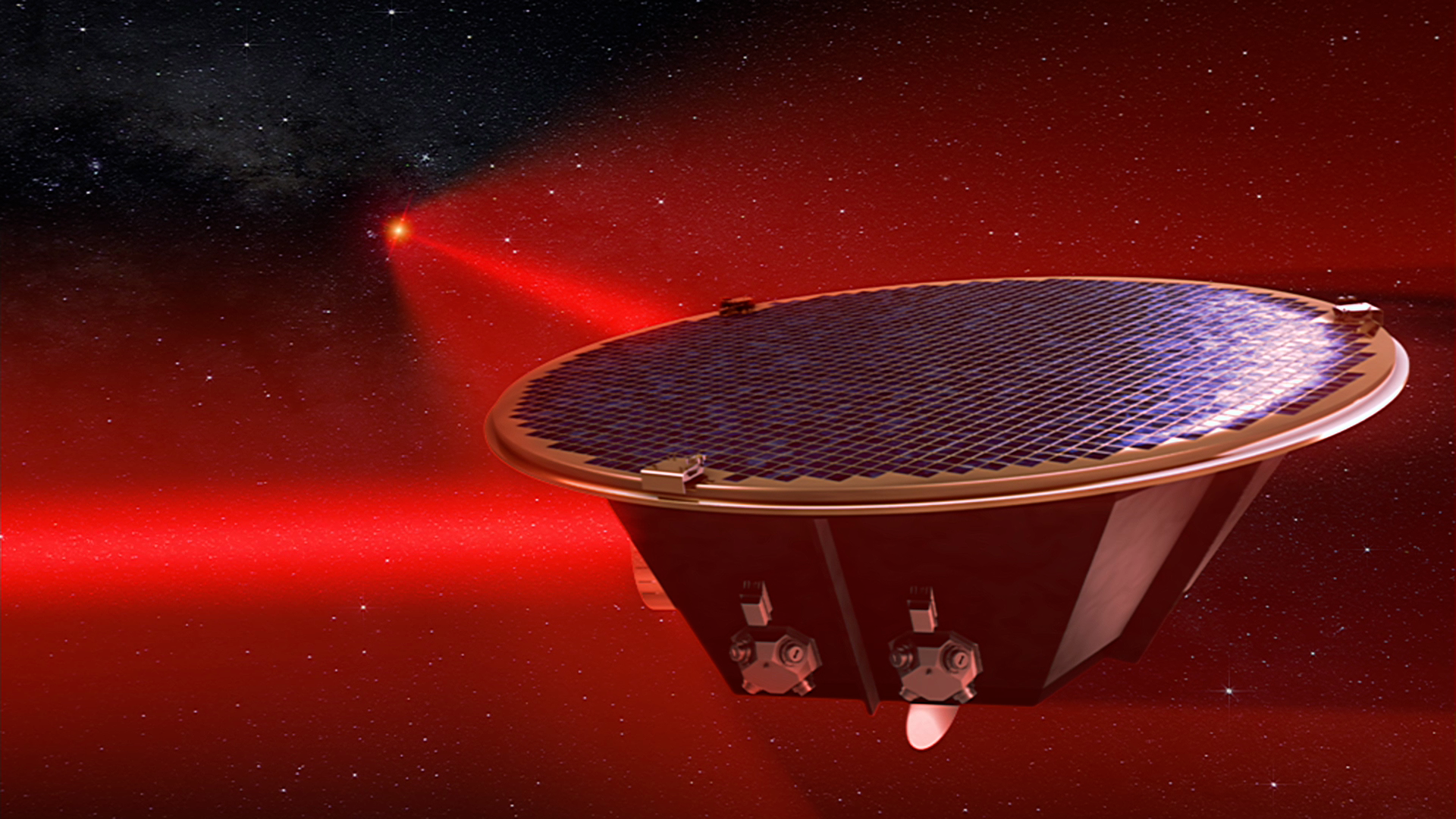
There are two solutions for this: increase the laser power (in LISA they are 1W) and increase the initial beam size. The larger the beam at the beginning of the path, the smaller its divergence. For example, if you make the initial diameter of the beam not 1 mm, but 1 m, the distance on the satellite will be “only” 5 km. But this is already enough to get several picowatts on the distant cube. This is exactly what is done in LISA: the diameter of the beam emerging from the satellite is is it specially made large enough so that enough light reaches the other satellite?
Stop, the most attentive of you will say. Several picowatts reach the cube, but the light has to be reflected and then fly back?! Something doesn't add up here.
And they will be absolutely right. The fact is that I deceived you a little when I compared the operation of LISA with a conventional interferometer. In fact, the light is not sent back after being reflected from the cube. Instead, it is combined with a local laser beam.
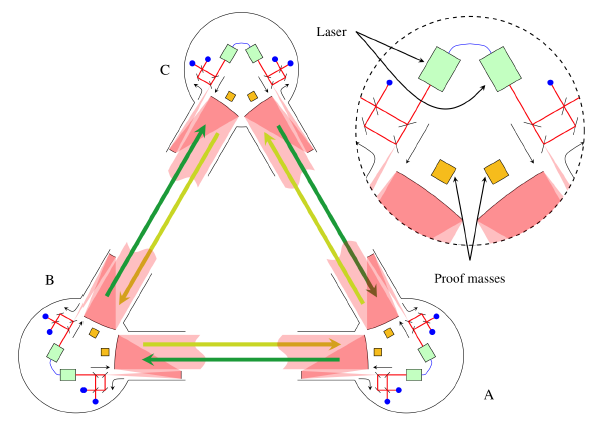
Step by step: The laser beam is split into two parts on the satellite. One part is reflected from the cube and sent to another satellite, and the second part interferes with a beam from another laser coming from another satellite (also previously reflected from the cube). Thus, the two beams are “connected” to each other through local interference. The signal about the gravitational wave turns out to be “encoded” in the beam, which is reflected from two cubes. In LIGO we could literally see the signal with our eyes as it exited the photodetector
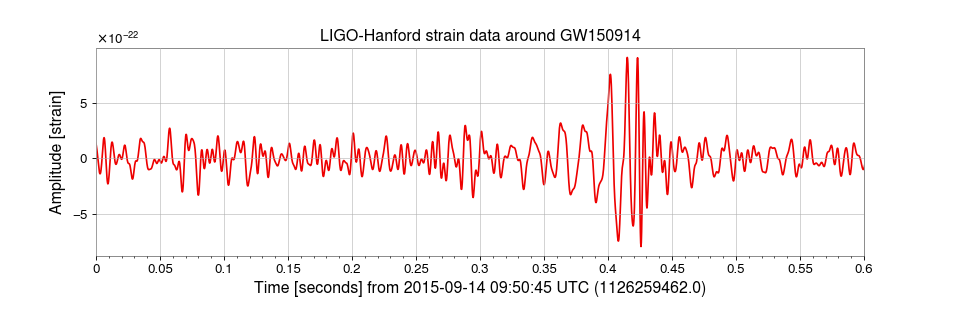
This will not work in LISA: signals will have to be extracted from data analysis.
The important point here is that, unlike LIGO, there is no Michelson interferometer with two long arms, but interferometers with one very long and one very short. Entire interferometers look like this:
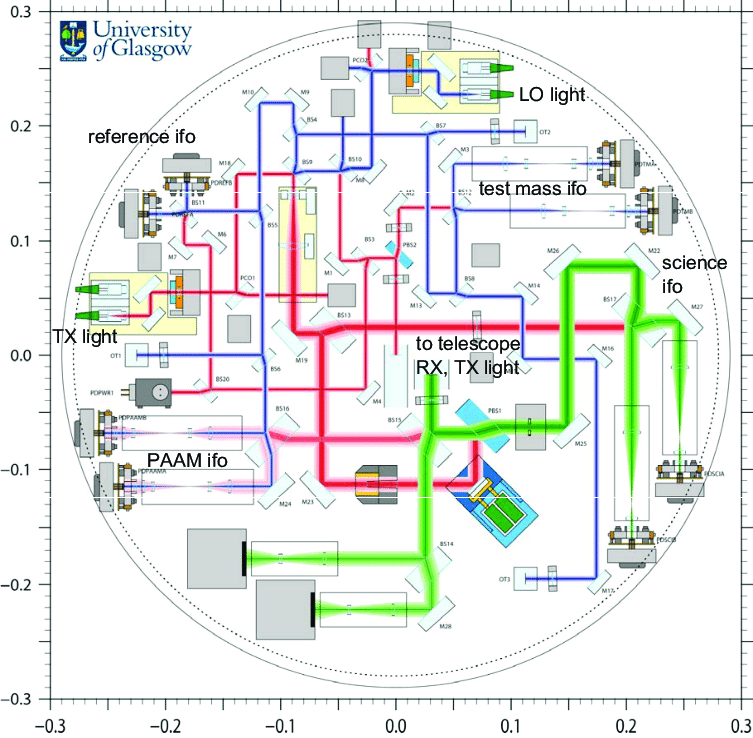
Can you imagine? All this should work somewhere far in space, and also catch gravitational waves. What if one mirror moves a little? In LIGO, the light beam is constantly adjusted to compensate for small movements of the mirrors. What can we do here?
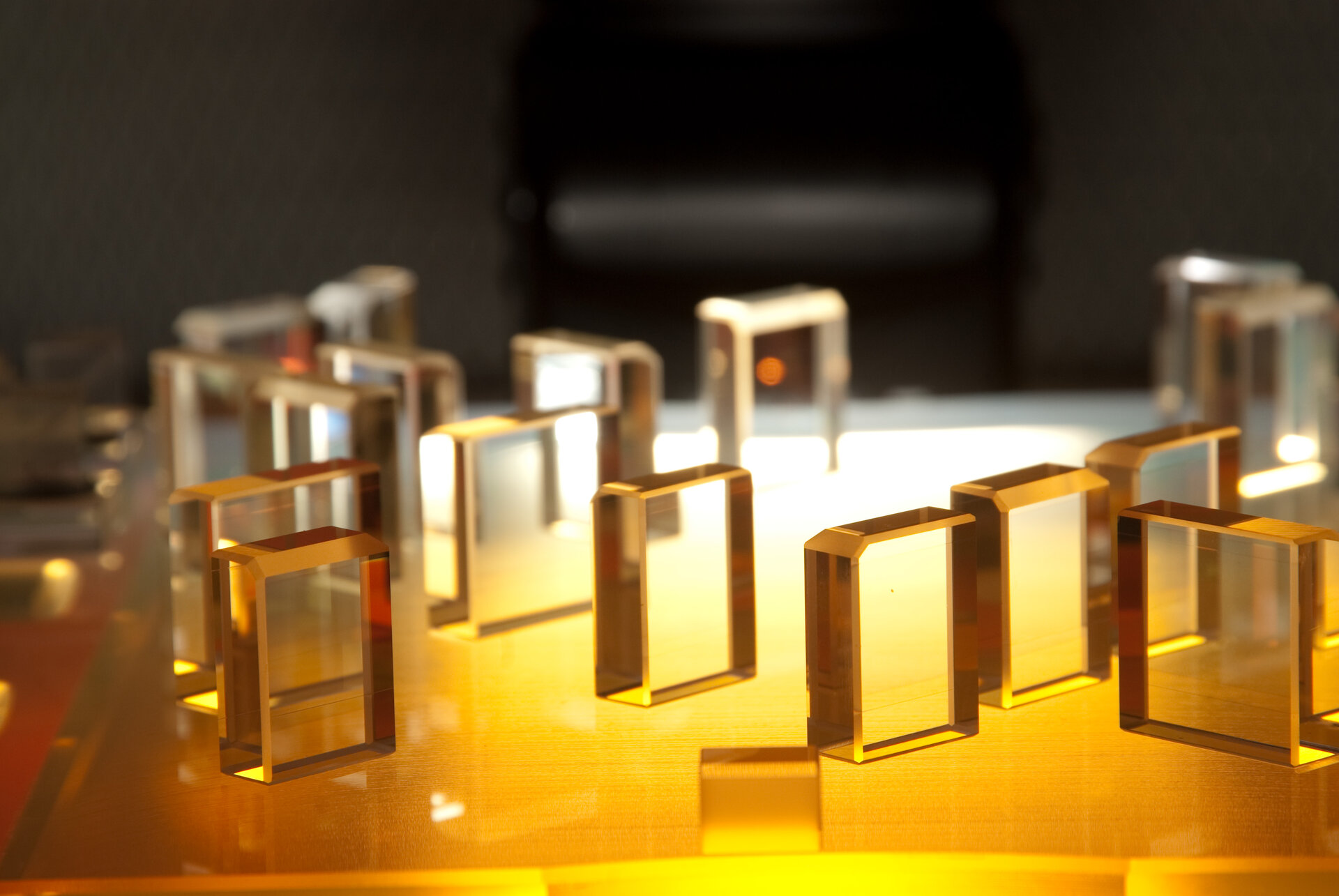
Like that. The entire optical installation consists of monolithic glass, to which the mirrors are glued with glass glue. They gather in clean rooms, where everyone wears protective suits and there is not a speck of dust, using a special positioning system that places the mirrors in place with great precision. The result is an almost monolithic structure, so that the mirrors do not move relative to each other. I used to work in laboratories where these optical installations are created – the complexity and thoughtfulness of the process is amazing.
But why am I, I promised to convince you that this is impossible, but I’m telling you the opposite. Let's discuss the difficulties further.
Laser noise
In conventional LIGO-type interferometers, the role of two long (and equal in length) arms is not only (and not so much) in signal detection. One shoulder would be enough for this. Two arms are needed to compensate for laser frequency fluctuations.
The fact is that the frequency (wavelength) of any, even the most stable laser changes slightly – this is called frequency noise. To some this may be more familiar as laser bandwidth. This is a fundamental effect associated with light generation (and is ultimately quantum).
So this noise, even in the best lasers, is many, many, many orders of magnitude higher than any signal that we want to register. But fortunately for us, in interferometers this noise can be eliminated by equalizing the length of the two arms. How it works? Let's select a small “piece” of a light wave that has slightly changed its frequency. It is divided into two at the beam splitter, the two parts of this piece go the same way in the arms and return to the central beam splitter. There they interfere destructively and no noise leaks through to our photodetector.
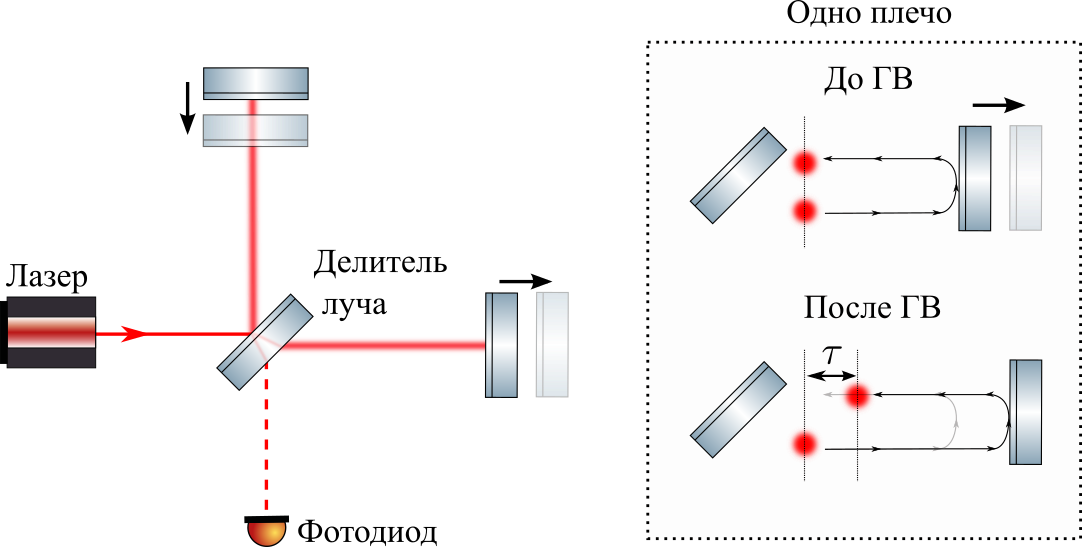
Now imagine that one shoulder is much smaller than the other. The piece is divided into two, but then one travels a very short distance and returns to the beam splitter much earlier than the second. There is nothing for it to destructively interfere with and it climbs out directly onto the photodiode, completely blocking all gravitational waves.
What to do? Cunning data analysis comes to the rescue when a virtual interferometer with two long arms is created. This is called time delay interferometry, and a detailed explanation of this is perhaps too complex for this article. Those interested can dive into the topic at this or this article.
The main thing is that this approach makes it possible to reduce the effect of laser noise by many orders of magnitude, but requires complex data analysis.
Noises
Not only do gravitational waves change the distance between the cubes, but also a lot of noise from different sources that either physically changes the distance or masquerades as such a change. I will list a few of the most important:
Measurement noise: all kinds of noise associated with the measurement process: laser noise, electronic noise of photodiodes and shot noise.
Thermal noise: Even though the satellite is in space, all elements vibrate slightly due to the Brownian motion of molecules in the material. This thermal noise occurs on cubes, mirrors and other surfaces.
Actuator noise: remember that the cubes are kept at a constant distance from the insides of the satellite? The electric field that is used for this fluctuates slightly, resulting in additional forces on the cube.
Engine noise: The position of the satellites must be constantly adjusted, otherwise they will fly apart or turn away from each other and no triangle will be formed. To achieve this, the jet engines constantly maintain the desired orientation. Of course, the thrust of the engines is not constant and leads to fluctuations in the position of the satellites.
Residual gas: In space, although there is a vacuum, there can be a few particles of different gases. These particles collide with the cubes and push them.
Guidance system: To maintain the orientation of the satellites, a special guidance system is used. It is also not ideal, which leads to errors.
Each of these noises requires optimization and special design.
Of course, these are only fundamental difficulties; there are also a lot of purely technical ones: power and fuel, isolation from external influences, data processing…
I don’t know about you, but this project has always seemed purely fantastic to me: we have difficulty detecting gravitational waves on Earth, but now we need to launch it into space! But fantasy sometimes becomes reality.
LISA Pathfinder
In 2015, the LISA Pathfinder satellite was launched – a prototype of a full-fledged LISA.
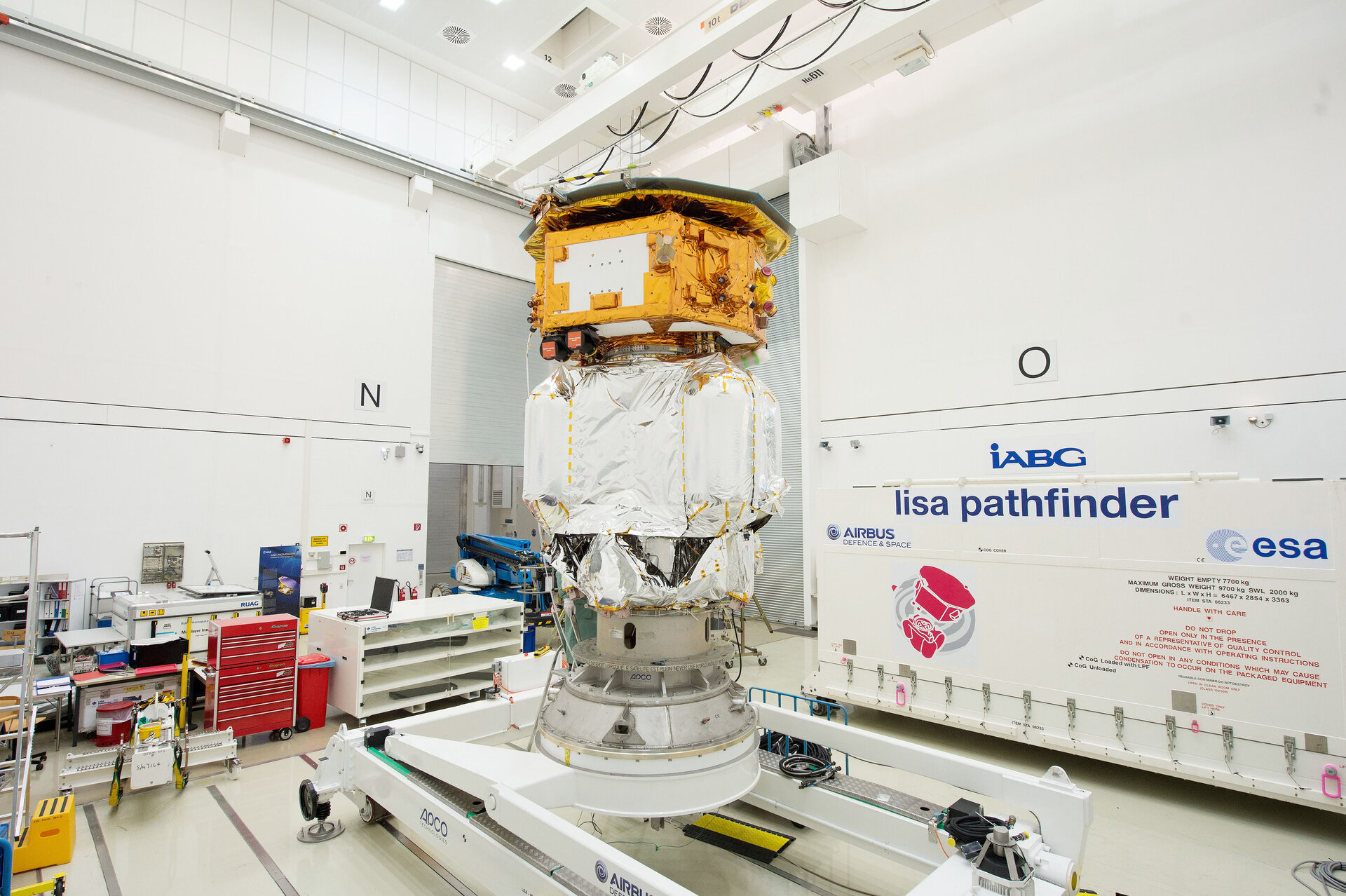
The goal of the project was to test the measurement process. Two cubes were placed inside the satellite (which, just like in LISA), flew “inside” separate cameras. In fact, there was the same interferometer as in LISA, only inside one satellite.
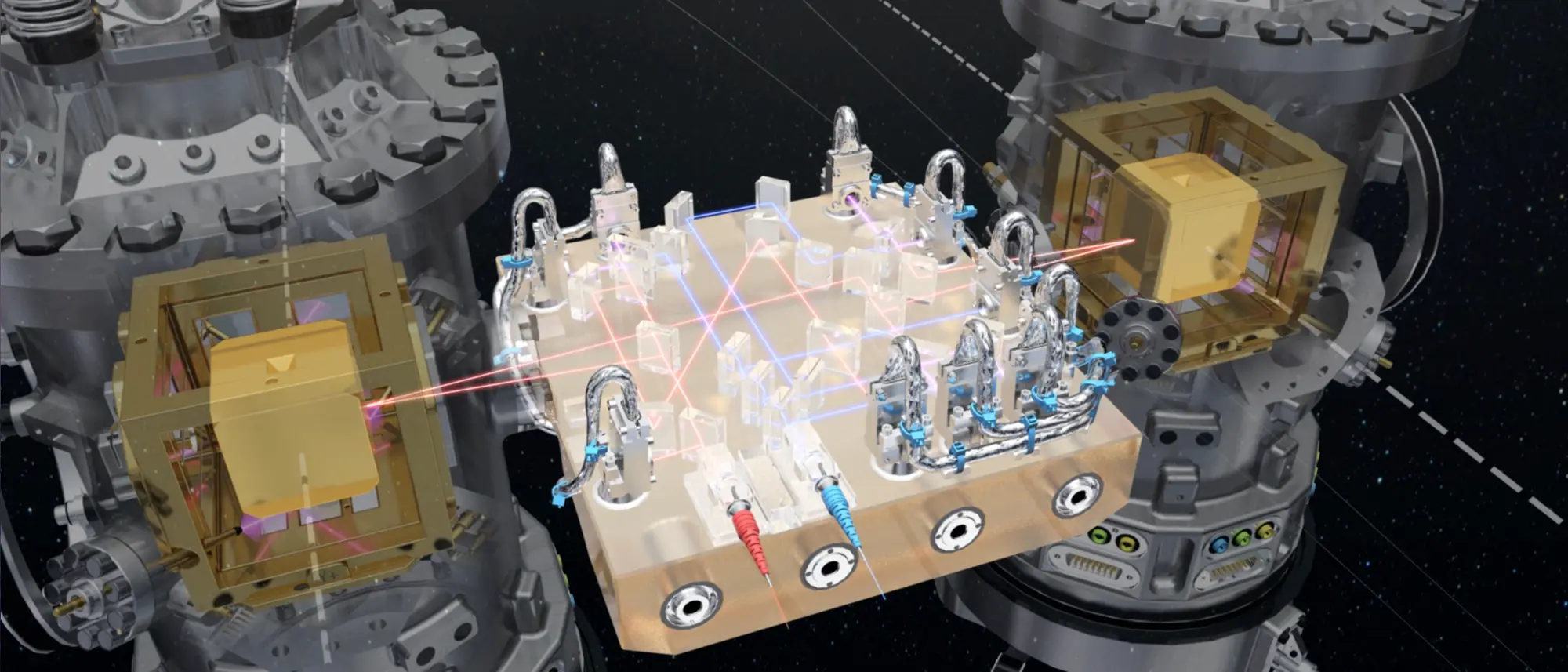
The satellite was launched to the Lagrange point, where it hovered for about a year, checking the reliability of the systems. The main goals were:
Checking the operation of the interferometer with test masses
Controlling a satellite without creating additional forces on the cubes
Testing technologies for strength (can a laser work in space? – it can!)
The mission accomplished all this not just perfectly, but exceeded all expectations. Look at the graph of sensitivity (i.e. the minimum strength that a detector can detect when limited by different noises at different frequencies):
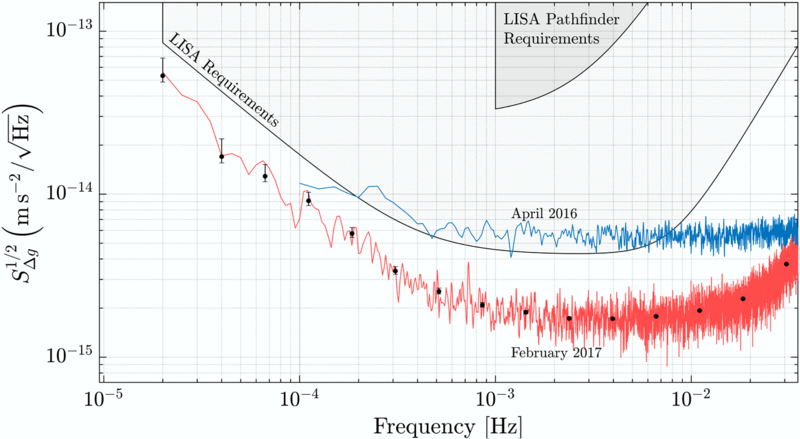
This phenomenal success proved that LISA could work and opened the door to all the financing under the sun.
What will happen next?
Following the success of Pathfinder, the European Space Agency fully sponsored the LISA project. Now it is being prepared in full: the launch is scheduled for 2035. The mission itself is designed for 4 years (and if you're lucky, up to 8 years, depending on component wear and fuel consumption). If successful, we will be faced with a huge amount of data that will need to be processed somehow. The biggest problem with this is the overlap of different sources on top of each other. LISA will see everything at once: mergers of galaxies and supermassive black holes, and the stochastic background of gravitational waves, and mergers of compact objects, and many at the same time. Scientists will have to figure out how to separate them and how to obtain the necessary parameters from these observations. It’s good that there are still more than ten years to learn this properly.
What about other space detectors?
But a legitimate question may arise: LISA will catch waves with a frequency of up to 1 MHz maximum, and the minimum frequency for LIGO/ET is on the order of several Hz. What about the window between 1MHz – 1Hz? Should it also be full of interesting sources?
Indeed, here again space detectors can come to the rescue, but with a smaller arm size. There are two leading projects here: Chinese TianQin and Japanese DECIGO.
TianQin is essentially a copy of LISA, only with a shorter arm length (about 100,000 km), and it will fly in Earth orbit.
DECIGO is a little more interesting: its arm length is only 1000 km, it also flies in Earth orbit, and it uses a real interferometer not only with long arms, but also with optical resonators in the arms, just like in LIGO.
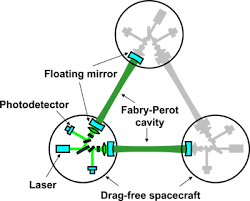
While these projects are in the early stages of development (although TianQin is developing at a breakneck pace – the Chinese probably want to overtake LISA).
What will happen is unknown, but the mid-30s will clearly be a boom in gravitational wave detectors: the Einstein Telescope and Cosmic Explorer will be launched on Earth, LISA and, possibly, TianQin will fly in space. There's so much we can learn about the Universe—it's dizzying with the possibilities! All we have to do is wait…
For those interested, my other materials on gravitational waves:
You can follow my scientific adventures in the channel “Homeostatic Universe“, where I write about interesting things about the Universe, quantum physics and the life of a scientist in general.
