A little about Hawking evaporation
Despite the great interest in this topic, many sources and almost all popular science incorrectly explain this phenomenon. The reason is that S. Hawking himself initially gave an incorrect heuristic interpretation of his discovery, namely: using a combination of quantum physics and Einstein’s theory of gravity, he explained that the spontaneous birth and annihilation of pairs of particles can occur near the event horizon. Typically, a particle and its antiparticle are born from a quantum field for a very short time, after which they are immediately annihilated. But sometimes it can happen that one particle falls beyond the event horizon into a black hole, while the other escapes. The escaped particle, having moved away from the opponent to a distance greater than the Heisenberg distance, turns into a real one and we can detect it as radiation. The energy of real particles is equal to the mass multiplied by the square of the speed of light when the particle is at rest, and increases as the particle begins to move; therefore energy can never be negative. Thus, if the real particle that escaped from the black hole has positive energy, and the total energy of the original virtual pair was zero, then the particle that fell into the black hole has negative energy. And when that particle falls into a black hole, the total mass of the black hole decreases.
According to Hawking himself, this process should ultimately lead to the evaporation of the black hole. However, even with the naked eye, serious shortcomings of this explanation are visible. There are many scientific articles pointing out the errors of this interpretation, for example, we can recommend the brilliant article [1] however, our goal is to provide a more correct explanation for Hawking evaporation.
First of all, let us recall some information about the behavior of time-space in the vicinity of a black hole. An external observer monitoring processes near the event horizon of a black hole sees that particles falling onto a black hole will never reach the event horizon in a finite time. This is because the gravitational pull of a black hole is so strong that particles falling into it slow down very much as they approach the event horizon.
According to general relativity, when an object approaches the event horizon, time slows down for it from the point of view of an external observer. This means that for an external observer, the fall of a particle to the event horizon takes an infinitely long time, since time for this particle slows down to infinity. Thus, from the point of view of an external observer, particles falling into a black hole will never cross the event horizon in a finite time.
Let us present the corresponding calculations. (In the future, all texts in italics can be skipped, as they require sufficient mathematical preparation).
For simplicity, let us take the most extreme case of the radial motion of a particle towards a black hole and the equation of its geodesic:
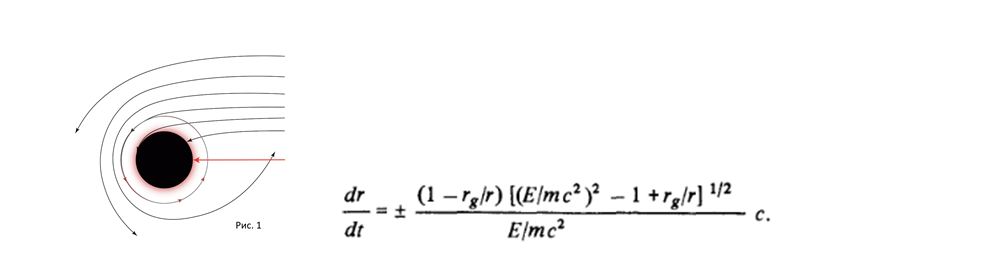
Time of movement of a particle from r = r1 to the event horizon r = r g is determined by integrating this equation, and the resulting integral diverges, which indicates an infinite time for a particle to fall onto a black hole [1].
Thus, the structure of regions inside a black hole depends crucially on the fate of the black hole in the infinite future of the external observer, for example, on the final state of evaporation of the black hole, on possible collisions of the black hole with other black holes, and on the fate of the Universe itself. It is clear that theorists feel very uncomfortable and are doing their best to avoid such a formulation of the question, transferring their research to the internal time of both the falling particle and the black hole itself. These delights seem to be counterproductive, since in no way can they affect the processes of the external Universe, since the lifetime of the Universe is less than the time the particle reaches the event horizon. And besides, “unsolvable” questions arise again, such as:
a) interpretation of the process of transformation of space-time on the event horizon and beyond it, since the properties of space become temporally similar, and the properties of time spatially similar,
b) transformation of matter and energy beyond the horizon
c) the existence in this “new space” of ordinary matter and energy.
Besides, falls There are no mass and massless particles beyond the event horizon in a finite time, and the evaporation of a black hole occurs in a finite time [2]:

Further everywhere: ℏ – reduced Planck constant, c – speed of light in vacuum, G – gravitational constant, M – the mass of the black hole.
We will present a mechanism for black hole evaporation that does not use an event horizon, thereby solving these problems.
Our interpretation uses an approach similar to the Schwinger effect [3] the essence of which is that the particles of a spontaneously created virtual pair are accelerated in opposite directions by an external electromagnetic background field. If the particles separate far enough beyond the time allotted to them by Heisenberg's uncertainty principle, that is, if the virtual particles gain enough energy at a Compton wavelength distance to obey the relativistic energy of the momentum ratio E2 = m2 + ⃗p2, they become real.
In spacetime, curvature will play the same role as the electric field strength in the Schwinger effect. As one approaches the event horizon, the curvature of space rapidly increases, and tidal forces become increasingly uneven. Because of this, some virtual couples will be separated by these forces and become real. Then some of them will escape from the black hole, some will annihilate and some will be captured again, in accordance with the standard escape fraction of massless particles born near the black hole (see Fig. 2)
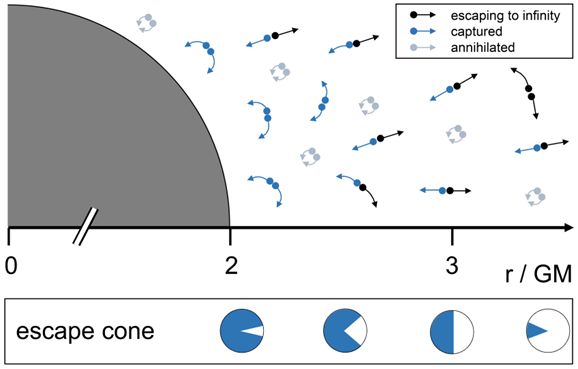

Let us consider the formal representation of the birth of virtual particles in the region of a black hole from the event horizon to infinity, and later we will provide a brief mathematical justification.
As mentioned above, virtual particles are constantly being created in regions below the Heisenberg size. In ordinary flat space, particles do not have time to fly apart and annihilate, but if space is strongly curved, then this helps the particles to fly apart. Calculating statistics using the introduced approach, it is determined that the frequency of particle production events is highest at short distances from the event horizon, while the probability of escape is highest at large distances (scale escape cone in Figure 1).
In addition, the mathematical apparatus makes it possible to calculate the radial profile of the formation of particles going to infinity per unit time of an external observer: from a sharp drop towards the event horizon to a power-law decrease at large distances. The highest runaway particle production density is observed at 2.32GM, while the highest number of particles per spherical shell is approx. 2.47GM, which is approximately halfway between the event horizon and the circular photon orbit at 3GM.

Next, the energy flux of the created particles at infinity is estimated and the energy of the gravitationally created particle is derived.
Comparing the number of particles and energy flow with the Hawking case, it is established that both effects are of the same order. The presented pair production mechanism itself does not explicitly use the presence of an event horizon and does not contradict the previously introduced restrictions.
It is clear that if there is radiation without an external energy source, this means that space-time is not stationary. Therefore, the black hole actually evaporates.
The last statement requires special attention. It says that in the region of space containing a black hole there is a decrease in the gravitational field and a “straightening” of space. This means that there is a decrease in the mass of matter in this region and, therefore, due to the adiabatic principle, a decrease in the mass of the black hole itself.
In addition, due to the restriction on the penetration of particles beyond the event horizon by the time of the external Universe, we avoid the information paradox in the following way. The concept of information paradox is closely related to the unitarity of quantum operations, and only a violation of unitarity leads to the loss of information. After gravitational collapse, from the point of view of the external Universe, no changes occur in the black hole, including no transformations (neither unitary nor any other). No matter or energy enters a black hole. The mechanisms of particle creation and escape described above, which ensure the evaporation of black holes, are unitary. Consequently, only unitary operators are present everywhere and there are no prerequisites for creating an information paradox.
Roughly speaking, quantum physicists need unitarity; without it, the entire edifice of quantum physics will collapse. All other questions about information do not bother anyone. For example, all processes in the macrocosm are accompanied by an increase in entropy (according to the laws of thermodynamics), which will eventually absorb all the information – so what? Physicists don't care about this information, that's why there's no talk. In fact, this paradox is worthless, does it disappear, does it burn out, is it restored – what does it change? Nothing. But unitarity is, yes. This is sacred.
Particle birth.
To build a mathematical model, the idea of a single-loop effective action will be used. In quantum field theory, the effective action can be used to describe the behavior of a field on some energy or time scales, ignoring details of scales that are too small or too large for the phenomenon of interest.
Let's turn to Figure 1. To implement the visualized ideas, we need to introduce a map of the region near the black hole, simulate the birth and behavior of particles, and collect statistics of escaped particles. For these purposes we will use the following concepts from quantum field theory: classical action W (or effective action) and single-loop effective action WE. Physical meaning W is that it represents a map of the area in Figure 1, and characterizes the behavior of particles on this map, Weff is responsible for the virtual particles on this map.
In quantum field theory, the effective action can be used to describe the behavior of a field on some energy or time scales, ignoring details of scales that are too small or too large for the phenomenon of interest. The action W of the electromagnetic field is a scalar function of the fields Aμ (vector potential) and can be written as a Lagrangian integral over the entire space-time. It describes how the electromagnetic field interacts with charged particles and with itself. For example, the classical action of W for the interaction of a charged particle with a background electromagnetic field. Let us assume that this particle has a charge q, and the background electromagnetic field is characterized by the potential Aμ and the electromagnetic field tensor Fμν. Then the classical action W can be written as follows:
W=−m∫ds+q∫Aμdxμ
where the first integral represents the action for the particle's motion in space-time, and the second integral represents the action for its interaction with the electromagnetic field.
Now let's move on to the single-loop effective action WE. This includes quantum corrections to the classical action, taking into account the interaction of the particle with virtual quanta of the electromagnetic field. The formula for a one-loop effective action can be written as the sum of the classical action and corrections in one loop:
WE =W+δW(1)
δS(1) is a one-loop correction to the action, which depends on the virtual quanta of the electromagnetic field.
Formally, the expression δW(1) includes the sum over all virtual particles that can arise in the background field. We will present it in the form of a formal path integral. Since we will study and search for radiation, and Hawking evaporation is radiation of an almost black body (sergo body), we will need formulas for the one-loop effective action in the representation of the thermal core. In this case
If denotes the action of an electromagnetic field WA WE – single-loop
effective action. Then: exp(-WE ) = ∫ DAe−W where the integral is taken
over all possible configurations of field A. Or Z=∫DAe−W where Z is a function describing the probability that a given field configuration A will be realized
The effective action can be expressed through the trace of the spread operator. In the one-loop approximation, effective action WE can be written as the trace functional of the propagation operator D. Formally, this is expressed as:
WE =−lnTrD. Here D is the operator of particle propagation in a given background field. By taking the trace of this operator, all possible loop diagrams are taken into account.
Let us consider the stationary production of real massless scalar particles by weak electric and gravitational background fields. Following the Schwinger technique [4]it is clear that the corresponding vacuum instability rate is encoded in the imaginary part of the one-loop effective action, which is defined as:

In Euclidean space with metric gEμν it is possible to change variables to a Lorentzian signature in the case of stationary space-time. The first line expresses the effective action in terms of a second-order elliptic differential operator for the scalar field H. The second line makes explicit the interpretation in terms of field excitations localized at the point x(τ), which would classically evolve according to H as a Hamiltonian operator during proper time s. In terms of path integral, all excitation trajectories that start and end at the same point are considered and weighted according to the classical action [5]:

In the Lorentzian analogue, a closed trajectory requires the field excitation to move back and forth in external time. It can be interpreted as the virtual birth and destruction of a particle-antiparticle pair (represented by light gray trajectories in Fig. 1). The sum of all particle-antiparticle paths, i.e. the path integral in equation (2) is called the coincidence limit of the thermal kernel. It can be decomposed into the purely classical contribution exp(−m2s) and the contribution of fluctuations. This allows us to obtain the imaginary part of the Lorentzian effective action for a massless scalar in the weak field limit, in the form:
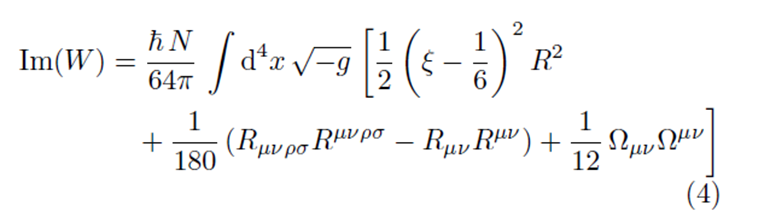
The scalar and Ricci tensor are denoted by R and Rμνand the Riemann tensor Rμνρσ . The curvature of the gauge field in electrodynamics has the form Ωμν = iqFμν/ℏ, which makes a contribution only for the charged, that is, complex, scalar. This effective action allows us to uniformly describe the Schwinger effect and our mechanism for the formation of gravitational particles. Equation (4) allows us to directly calculate the Schwinger effect, i.e. velocity density of vacuum instability taking into account the spontaneous creation of massless particles in a uniform electric background field in E, namely:

where Leff is the effective Lagrange density belonging to the effective action W. This result is consistent with the work of Euler, Heisenberg and Schwinger [2] on electron-positron pairs when generalized to a massless complex scalar field and when generalized to massless vector bosons in the non-Abelian Yang–Mills theory, in particular QCD
Now we can apply the same method to the quantum field in the curved spacetime of a black hole. We consider asymptotically flat, spherically symmetric and static Schwarzschild spacetime in (3 + 1) dimensions:

Due to Ricci flatness Rμν = 0 the only non-zero contribution to the imaginary part
the integrand of the effective action (4) is introduced by the scalar
Kretschmann Rμνρσ

When spatially integrated over the exterior of a black hole, which is a region of space-time causally related to external observers, the effective Lagrange function obtains

This predicts the rate density of particle formation events:

where the velocity refers to subsequent slices of the Schwarzschild constant time t, the proper time for a static observer at infinity.
With the help of this equation, the radial profile of the velocities of particles born from the gravitational field is ultimately constructed (Fig. 2). A more detailed description can be found in [6]
The popular description of Hawking radiation suggests that most particle production occurs at an infinitesimal distance from the event horizon. For our mechanism, we explicitly derive the radial particle production profile for evaporating black holes. Its peak is determined by the counteraction rate of creation of local pairs and the probability of escape (Fig. 1.)
The local, generally covariant expression for the imaginary part of the effective action in equation (4) actually indicates that this creation of gravitational particles does not depend on the choice of the vacuum state of the quantum field. This conclusion allows us to conclude that any massive gravitational object in the Universe, be it a star, galaxy, or nebula, evaporates over time.
Literature:
Joseph Polchinski “The Black Hole Information Problem” (2016). https://arxiv.org/abs/1609.04036
I.D. Novikov, V.P. Frolov “Physics of Black Holes”, Moscow, “Nauka”, 1986.
JS Schwinger, Phys. Rev. 82, 664 (1951).
With Hawking “Particle creation by black holes” “Communications in Mathematical Physics”, 1975.
Matthew J. Strassler “Field Theory Without Feynman Diagrams: One-Loop Effective Actions”, 1992, https://arxiv.org/abs/hep-ph/9205205.
Michael F. Wondrak, Walter D. van Suijlekom, Heino Falcke “Gravitational Pair Production and Black Hole Evaporation”, 2023, https://arxiv.org/abs/2305.18521